We Need To Take CO2 Out Of The Sky
To keep below two degrees, we'll need to dramatically reduce current emissions and simultaneously remove 10-15 gigatons of CO2/yr from the atmosphere by 2050. Read on for what that means, why, and how we might do it.
I wrote this to share some of my learning over the last few months with the goal of helping you, a non-scientist, bootstrap a reasonable understanding of the basics of carbon removal. No physics, chemistry, or climate background required. I got obsessed with climate as I started working on Stripe’s negative emissions commitment last year. This post was inspired by Longevity FAQ.
I wrote this in my spare time primarily to help my own learning. It’s definitely not perfect. Along with reading this, examine primary sources in my Negative Emissions Reading List, as well as the links throughout.
This post may contain errors large and small – if you find a meaningful factual error, I’ll correct it, credit you, and pay you $10 (cf). If you find typos, please let me know but I don't think I'll pay you for those. Overall, please let me know (Twitter, Email) any feedback or suggestions on how I could make this more useful!
Summary
Since the industrial revolution, people have been burning lots of stuff—more every year. From little fires in cars to big fires in power plants, we’re emitting greenhouse gases. We’re putting enough of these gases into the sky that we’re changing the thermal properties of the atmosphere, trapping more heat. This is the greenhouse effect, which is the main contributor to climate change.
Beyond power and transportation, there are less obvious emissions sources as well: cement production, steel production, agricultural emissions (soil nitrous oxide, animal methane), industrial and permafrost methane leaks, etc. You can explore the full range here.
Some sectors have clear paths to reduce emissions (electric vehicles, solar/wind/nuclear), some will be slower or more complex to decarbonize (air travel, industrial emissions, some agricultural emissions, methane).
Climate models describe how much warming we can expect in different emissions reductions scenarios. 1.5 degrees C is now an incredibly optimistic target that would require unprecedented reduction, 2 degrees is considered difficult but in reach, 3+ degrees would be a worst case scenario. These scenarios are described by "Representative Concentration Pathways" (intro, more detail). There's international agreement around 2 degrees C as maximum acceptable risk.
With that in mind; there are two general approaches to keep warming to below a certain level:
- Reducing emissions
- Removing previous emissions from the sky
If you remember one thing from this piece, it should be that we need to do both. Gone are the days where optimistic emissions reductions kept us below a 2-degree warming target.
To keep below two degrees, we'll need to dramatically reduce current emissions and simultaneously remove 10-15 gigatons of CO2/yr from the atmosphere by 2050 and scale that to about 20+ gigatons annually by 2100. Depending on how quickly we reduce emissions, the amount we need to remove from the atmosphere scales proportionally.
When I was little I looked at the space race and dreamed the world would rally around a huge science project again.
— Ryan Orbuch (@orbuch) January 8, 2020
10+ gigaton scale negative emissions is that! It’s urgent, necessary, hard, dramatic, all of it. It’s the defining project of our generation.
A quick sense of scale
Here's where we're at in terms of global temperatures:
Greenhouse gas emissions are described in units of tons. It’s hard to think about how much “a ton of gas” really is -- this is how big, at surface temperature and pressure:
Here’s a nostalgically-animated video visualizing a bunch of these one-ton balls in New York City.
If you’re in the US, you’re responsible for emitting about 19 tons of greenhouse gases a year. Wren has a nice calculator that asks you about your commuting habits, flights, and diet to estimate your total GHG emissions. Erika Reinhardt wrote a detailed guide on how you can reduce your emissions. With that in mind, see emissions per capita:
You may also hear people talk about “400 parts per million CO2” or similar. This maps directly to the amount of gas emitted: when emissions mix into the atmosphere, we reference concentrations of the emitted gas as a portion of the atmosphere. This is like stirring sugar into a cup of coffee. Climate modeling is based on these concentrations. For a bit more on how this measurement works, see Basic intro to units and measurement.
For context (thanks to Klaus Lackner for the following distillation), 1 ppm is worth about 7.5 Gigaton of CO2. But roughly half of it mixes into the surface ocean and some goes into the biosphere. So the net result is that it takes about 15 Gigaton of CO2 to raise the atmospheric concentration by 1 ppm. We raise it by about 2.5 ppm per year.
Basic intro to units and measurement
Measuring greenhouse gases
Greenhouse gases are released by burning stuff as well as the product of other chemical reactions in industry. Generally, we’re talking about mostly carbon dioxide (CO2), methane (natural gas) (CH4), and nitrous oxide (N2O). N2O and CH4 are more potent greenhouse gases, but they occur in an order of magnitude less quantity than CO2, so removing them is generally much harder. Methane also has a much shorter half-life in the atmosphere than CO2.
Here are the units you’ll usually see:
Tons of CO2
Literally just a ton of CO2 by mass. This is the most common unit. It's often unclear whether someone means metric or imperial tons, which is annoying.
Tons of CO2E
The E means “equivalent”, you’ll often see this when reading about offsets or macro-scale emissions comparisons. “Equivalent” means another greenhouse gas, normalized to the warming potential of CO2. As calculated, methane is about 28-36x equivalent (GWP 100), so 1 ton of methane would count as 28-36 tons CO2E.
(Update March 2: my original methane GWP value was incorrect, fixed now, thanks Ryan Barrett for flagging)
Tons of C
This is mostly used when talking about sinking carbon in plants and soils, and it just means we only measure the C in the CO2, which maps 3:11 by molar mass. (Because the two Oxygen atoms each have a mass of 16)
(Mega)tons, (giga)tons of any of these
Megaton = one million tons; Gigaton = one billion tons.
Measuring greenhouse gases when they’re mixed into the sky
The atmosphere is made of a mixture of gases:
Greenhouse gases are a super small part of it, so instead of describing tiny absolute percentages, we use these units to describe how much:
Parts per million (PPM)
- Used mostly for CO2
Parts per billion (PPB)
- Used mostly for CH4, CFCs, and other trace gases.
This is what we mean by methane being far more dilute than CO2 (and therefore unrealistic to capture) -- we need to measure it in parts per billion.
This sense of scale helps explain why removing CO2 is an expensive proposition, thermodynamically and hence monetarily: it’s an extremely dilute (parts per million!) gas in solution — we’re talking about separating a tiny fraction of the air from the rest of the air.
Finally, taking a step back: the sensitivity of this system is really incredible -- we’re talking about changing the lives of people around the world by adding less than a basis point to the absolute concentration of a trace gas. It’s wild.
Do we really need to remove CO2?
In the 90s, negative emissions were not mainstream apart from early research by David Keith, Klaus Lackner, and others.
There was some combination of optimism that the problem wasn’t so bad, that the world would decarbonize at a sufficient rate that negative emissions wouldn’t be necessary, and that a policy framework would force action (large scale carbon price/cap and trade, which still doesn’t exist worldwide). This was all combined with fears that negative emissions present a moral hazard by giving ourselves an “out” for crucial emissions reduction work. A good summary of the history is here.
In an attempt to hit a 2 degree warming target, the Paris agreement calls on countries to set “Nationally Determined Contributions”, or NDCs -- commitments to a specific amount of emissions reduction over the coming decades. But these commitments are not even close to enough:
There are two complementary scary things about this chart:
- All existing Paris commitments (“NDCs” in the above figure) don’t get us even close to a 2 degree trajectory
- Countries are not even close to on track to hit even these commitments. This is an incredible collective action problem — each individual country faces minimal/zero “official” consequences for failing to do so.
Here’s what the emissions gap looks like for a 1.5 degree target (source):
And for a 2 degree target (source):
Putting this all together is this figure:
Compounding the urgency, that research into negative emissions technologies (NETs) have been dramatically underfunded in proportion to their importance. To quote the National Academies (emphasis mine):
“...the federal government spent more than $22 billion on renewable energy research and development from 1978 to 2013. NETs have not received comparable public investment despite expectations that they might provide ~30 percent of the net emissions reductions required this century (i.e., maxima of 20 Gt/y CO2 of negative emissions and 50 Gt/y CO2 of mitigation in Figure S.1). NETs are essential to offset greenhouse gas emissions that cannot be eliminated, such as a large fraction of agricultural nitrous oxide and methane emissions.”
How to take CO2 out of the sky
For a more comprehensive but still accessible overview, I recommend Adam Marblestone’s Climate Technology Primer and primary sources in my Negative Emissions Reading List, especially the Summary section of the National Academies Report.
Stripe’s Negative Emissions Commitment blogpost includes a brief overview of these and other technologies as well as some important context on adoption curves.
Briefly: there are plant-based, mineral-based, and chemical options.
Plant based solutions leverage a plant’s capacity to capture carbon via photosynthesis and the energy of the sun. Solutions in this category include (re)forestation, soil carbon sequestration, algae/kelp farming, and bio-energy with carbon capture and storage (often referred to as BECCS, this is basically a biomass power plant that burns wood and then is fitted with a carbon capture device to handle the smoke aka “flue gas”).
Mineral-based solutions include speeding the weathering of naturally occurring rocks, e.g. Olivine.
Chemical solutions include Direct Air Capture (typically coupled with geologic storage, the capture aspect is often referred to as DAC), where a big machine sucks CO2 out of the air; providing gaseous concentrated CO2 that can then be injected underground for permanent storage.
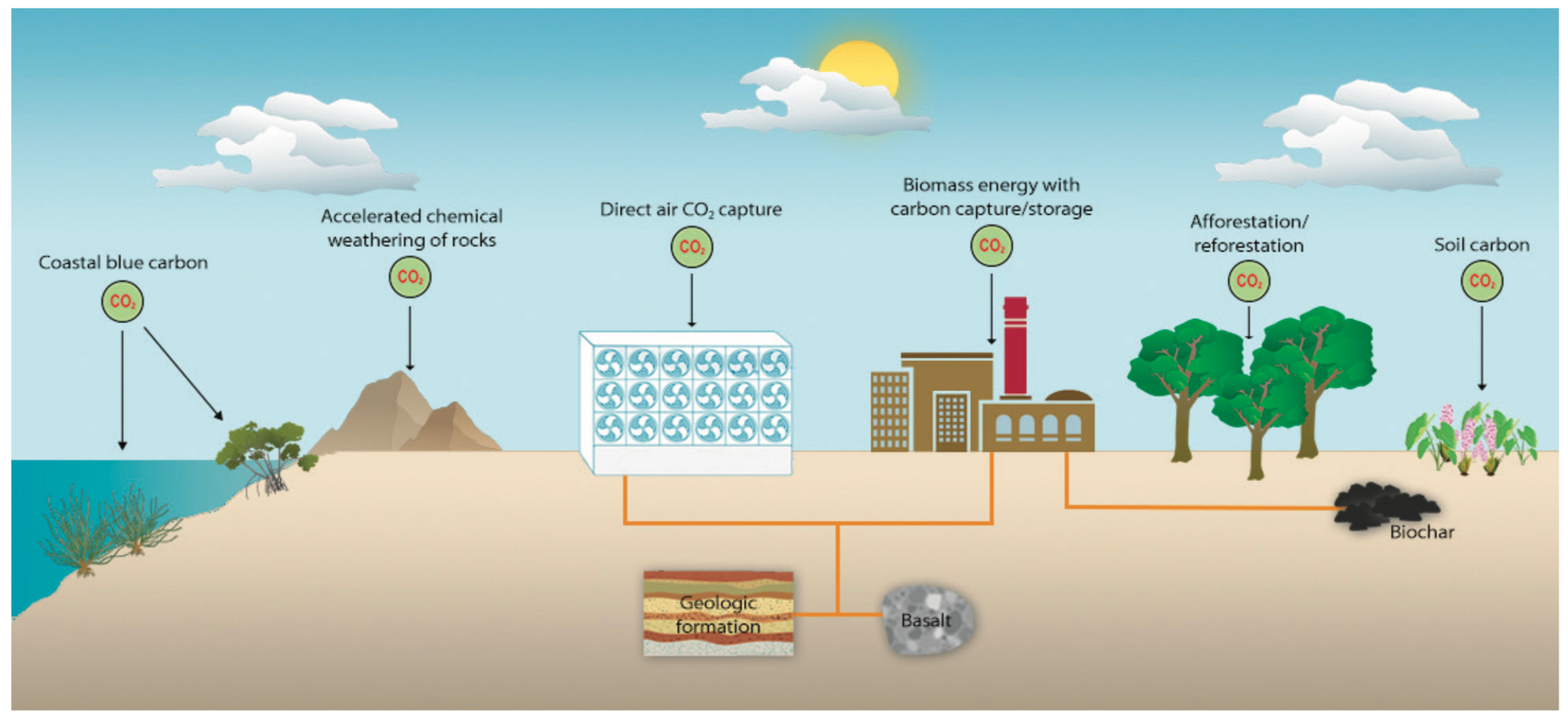
These solutions all have different technical tradeoffs, potentials for scale, costs, adoption curves, and more.
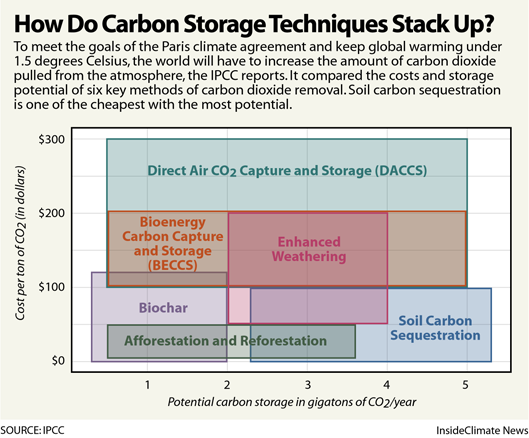
Overview of approaches
Trees and forests
Trees and plants are incredible at carbon capture, doing so for “free” via the energy of the sun despite how dilute CO2 is in the atmosphere. The CO2 trees absorb doesn’t magically go away. Instead, the C literally becomes part of the biomass of the tree. When a tree burns down, the carbon it absorbed is released right back into the air. For permanent sequestration, we’ll need to do something with the biomass of the forest. This could include making it into wooden building materials, biochar fertilizer, or dropping the biomass in the ocean. Or, if the forest is well managed, it can grow for centuries; and we can consider the sequestration “durable” as long as that management persists and is monitored.
To understand how much carbon is captured, scientists build models that relate easy-to-measure physical properties like diameter and height to the actual above-ground dry weight of a tree, about 50% of which is carbon. These are known as the allometric equations. More modern techniques leveraging remote sensing and LIDAR are being explored to do the same thing with fewer ground measurements.
Forests take up land that may be more economical if used for other things. Forests are dark colored and may actually decrease albedo (light-colored-ness -> heat reflectance from the sun), somewhat decreasing their utility (paper is here and here’s a lab working on albedo). There may also be impacts on the biodiversity of the ecosystem the land would otherwise be used for.
For more detail, I recommend the Rocky Mountain Institute’s overview report on land-based negative emissions solutions, as well as WRI’s land carbon removal overview.
Direct air capture (DAC) + sequestration
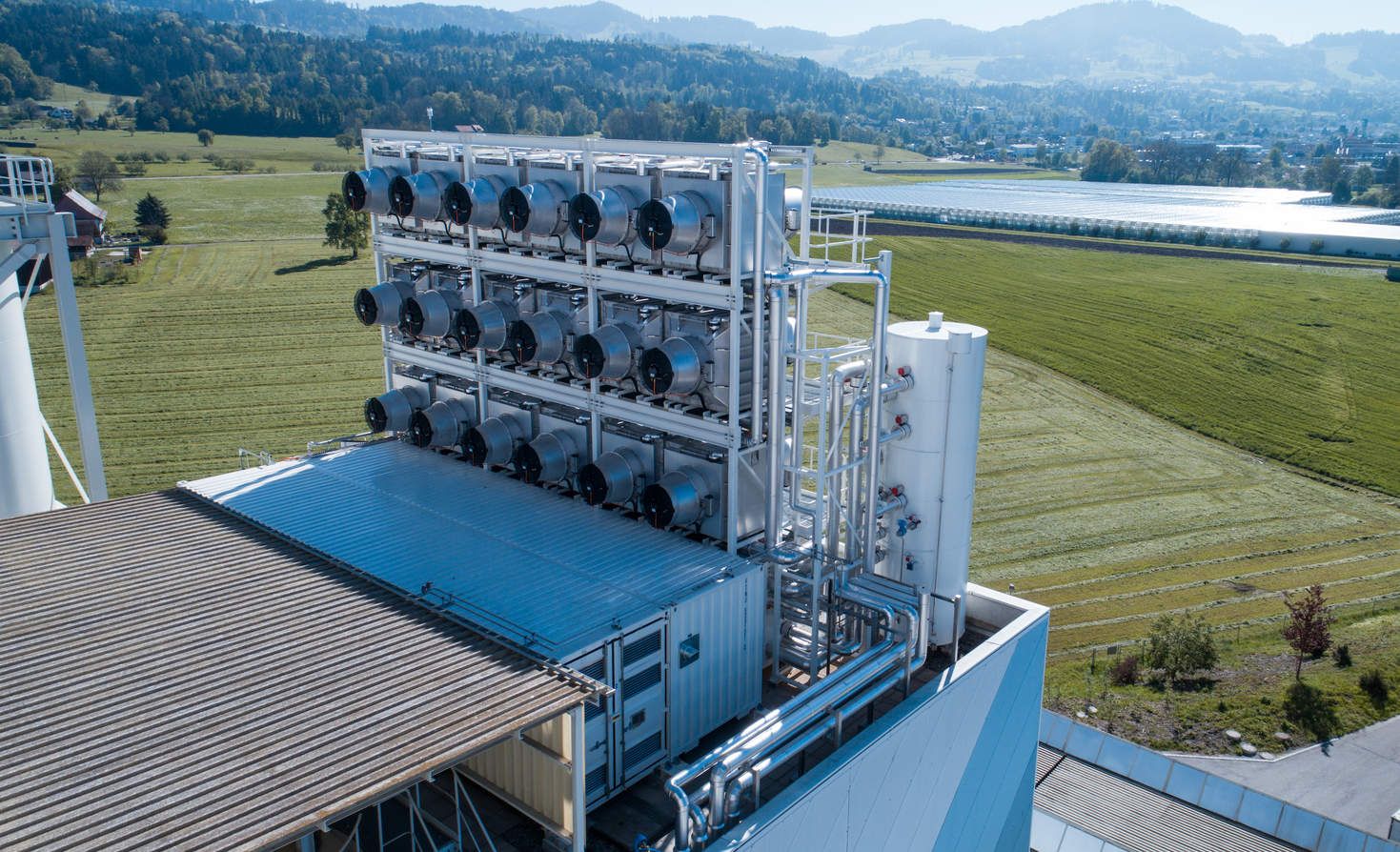
Traditional direct air capture facilities pass atmospheric air through a “sorbent” that absorbs, concentrates, and then controls release of CO2. For a detailed but still accessible overview of the field, see The Role of Direct Air Capture in Mitigation of Anthropogenic Greenhouse Gas Emissions.
Companies currently working on this include Climeworks, Carbon Engineering, and Global Thermostat. Because CO2 is so dilute (about 0.04% in atmospheric air), this process is energy intensive (22kJ/mol). Hence, Lifecycle Analysis is very important. For an example methodology, see Keith 2018 which describes Carbon Engineering's technical approach.
There are also some new DAC approaches, currently in the research phase, e.g. electro-swing adsorption (news article with a good summary, source paper). These are promising but quite early!
In any case, the result of DAC is pure gaseous CO2, which can be either utilized or sequestered.
Sequestration
Sequestering it means permanently removing the CO2 from the carbon cycle, and literally reducing the concentration of CO2 in the atmosphere. This can be done by injecting the CO2 injected underground into an oil well, salt dome, or saline aquifer.
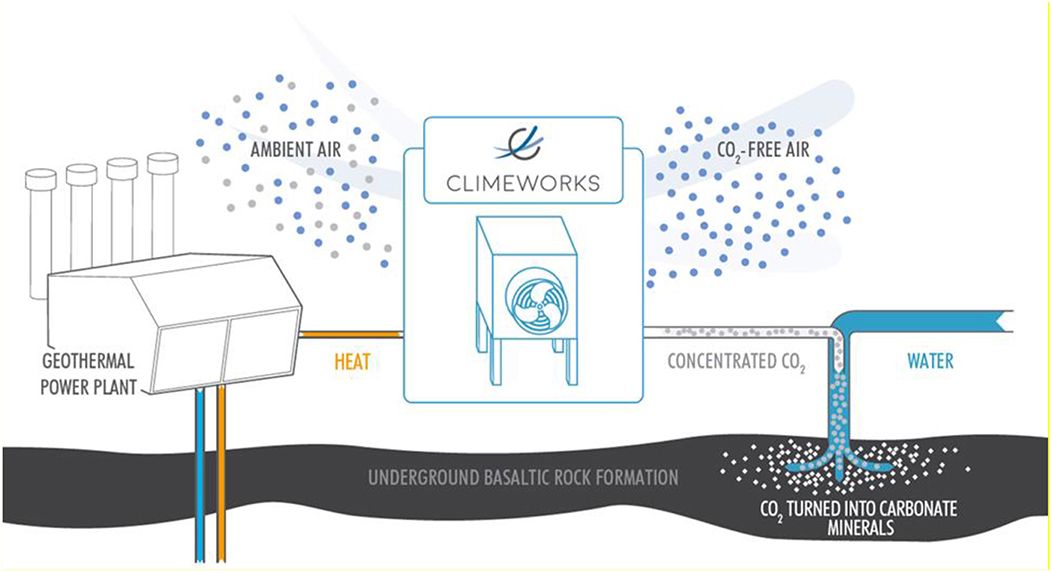
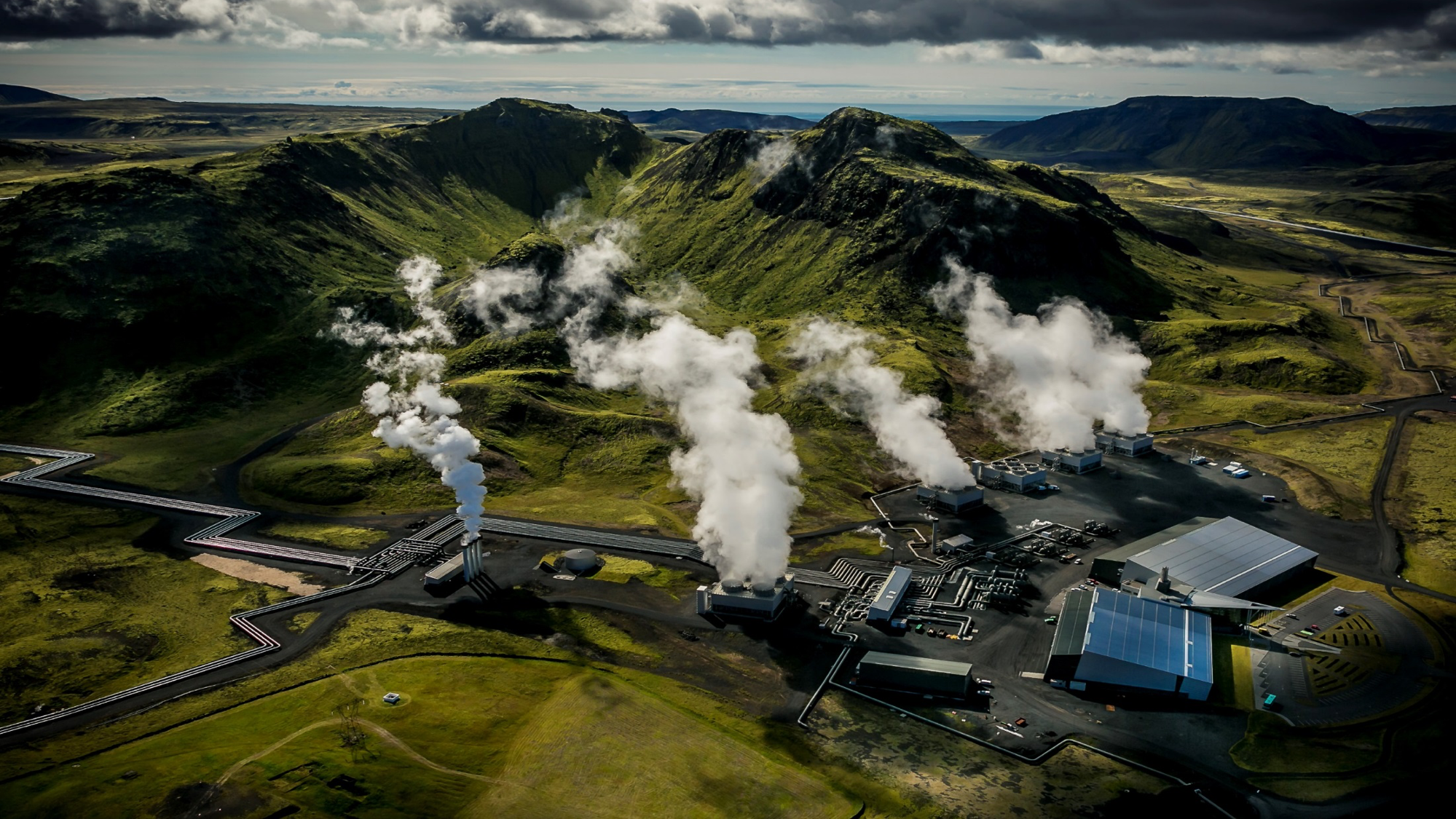
It’s fairly straightforward to measure how much CO2 is being pumped into geologic storage, and there are generally accepted practices in the oil industry to demonstrate secure storage. See the Geologic sequestration - "in situ" subsection of the Enhanced weathering and carbon mineralization section later down this blog post for more details.
Utilization
Alternatively, this CO2 can also be utilized. Some utilization mechanisms can be considered negative emissions/long-term sequestration, eg using the CO2 to cure cement. Other use cases include making fuels and chemicals. The fuels case is interesting, in that the emissions from the fuel wouldn’t cause an absolute increase in atmospheric CO2 like it would if you pulled the fuel out of the ground. In a sense, you’re burning and “unburning” the fuel via capture, concentration, and fuel reformation.
I'll note that none of these utilization solutions currently use direct air captured CO2 – they all capture the CO2 via the flue gas of a power plant or similar. Conceptually, they could just as well use a DAC-provided CO2 stream, it's just prohibitively expensive at this point.
Soils
Soil carbon sequestration methods aim to encourage modified agricultural techniques that increase the carbon sequestered in soil without reducing or otherwise affecting agricultural yield. For a deeper overview, I highly recommend Soil C Sequestration as a Biological Negative Emission Strategy.
These “regenerative” mechanisms include no-till farming, using cover crops, and reducing usage of nitrogen fertilizers can enable storage in soil of some of the plant’s carbon volume.
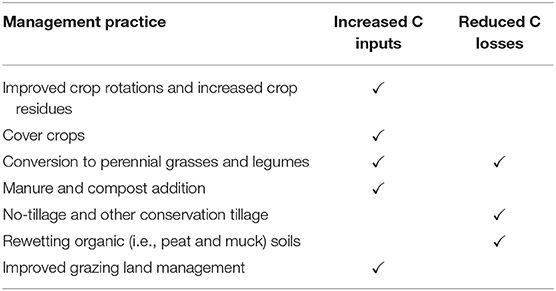
Measuring soil carbon can be complex and has high variance: there’s the carbon in the biomass of the plant roots, and then the carbon expelled by the roots that can become stable in the soil as "Soil Organic Carbon". Ground measurements are expensive (you take a core sample of the soil and stick it in a spectrometer, which is not a thing that farmers have lying around), and it’s still very early for soil carbon remote sensing.
Remote sensing is tough with soil because you don't just care about the carbon content of the topsoil, you care about the distribution of carbon vertically down the soil column for a couple feet – generally the deeper the carbon is, the more durably it's stored.
An alternate approach is "inverse modeling", where you try to extrapolate the soil organic carbon (SOC) based on the plant growth. More plant growth generally means more SOC, but isn't nearly a perfect correlate (precipitation, temperature, fertilizer use, crop type etc all confound, they also influence SOC but often with different statistical power).
Even if measured and modeled well, it can be hard to guarantee the long-term storage since farming and soil turnover practices could change in the future.
There are lots of labs working on soil stuff, if you're curious to learn more: Colorado State University is known for being a great soil program (they maintain COMET-Farm, the canonical farm soil carbon monitoring tool). CSIRO (the Australian govt research agency) is also very well respected and doing really interesting work in this field. Thanks to both of these groups for explaining soil things to me! Also take a look the Rocky Mountain Institute’s overview report on land-based negative emissions solutions, as well as WRI’s land carbon removal overview.
Doing things with biomass: Bio energy with carbon capture and storage (BECCS) and Biochar
BECCS
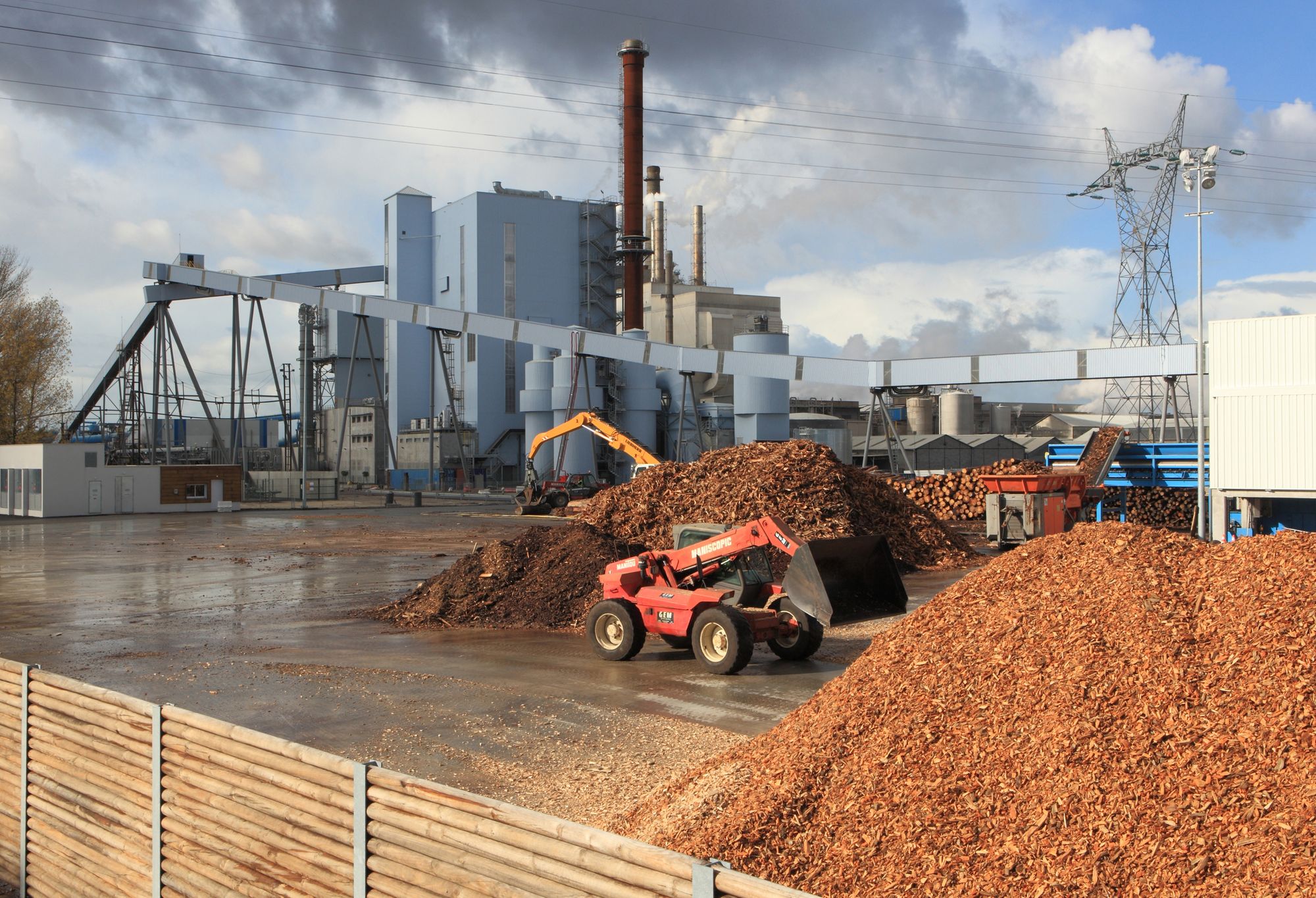
The idea of biomass power in general is that instead of burning coal or natural gas to turn a turbine, you burn biomass (which, because it's biomass, is made of carbon that used to be in the atmosphere).
Hence BECCS, as generally proposed, takes the “get the capture for free!” idea of plant-based carbon capture and combines it with "sequester the flue gas CO2" idea that’s often applied to the CO2 resulting from DAC.
BECCS strengths and weaknesses are similar to that of forests, where trees and grasses continue to be amazing at “free” carbon capture. The land use needed may be quite large, and likely more agriculturally intense. BECCS requires a very high volume of biomass (and hence has high sequestration potential, but is only currently deployed at very limited scale).
Depending on how you've combusted or pyrolyzed biomass, you either end up with a stream of flue gas from which you can concentrate the CO2 and do geologic storage/utilization/anything else you'd do with flue gas, or you're able to keep the C in the biomass via pyrolysis and output Biochar (see below) – this is what some new BECCS companies like Charm do.
Biochar
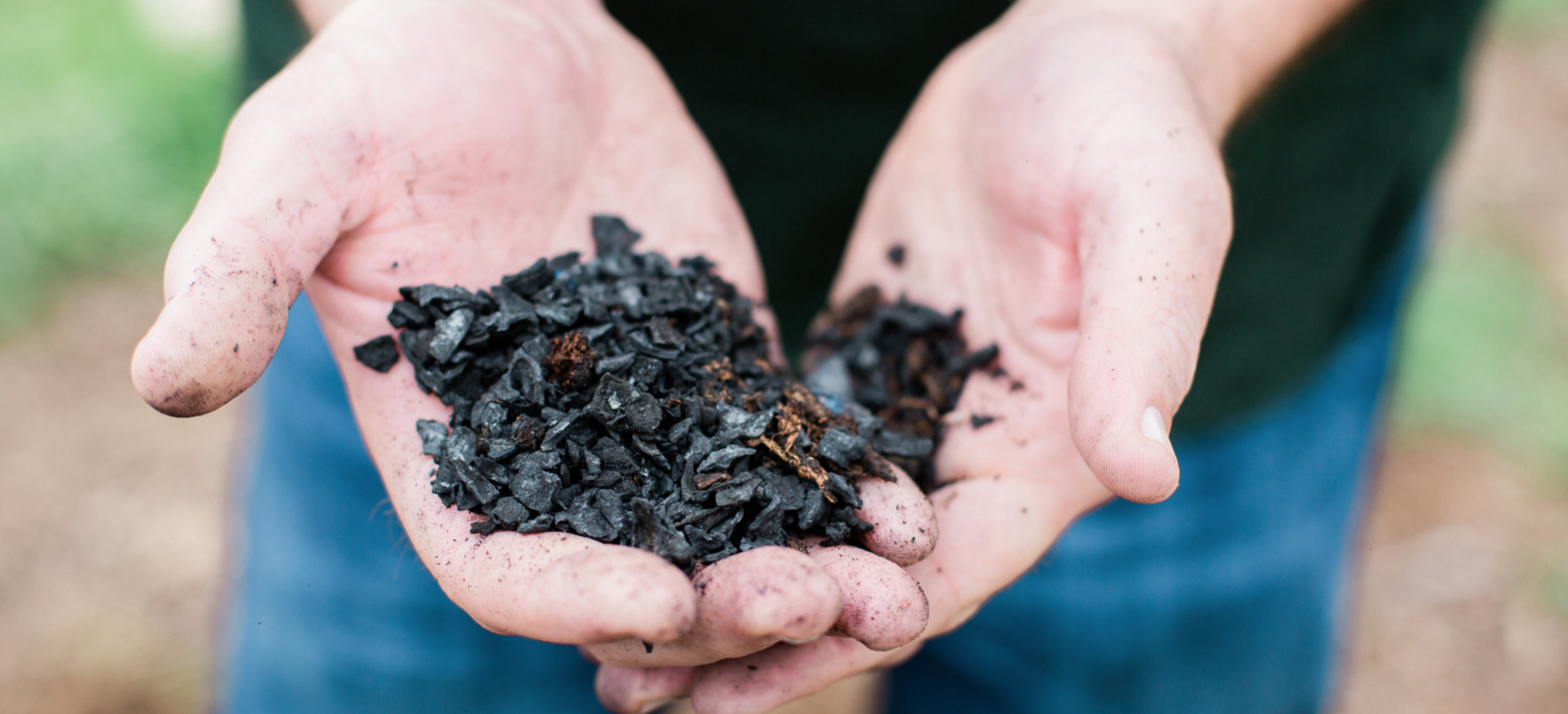
Another option is taking biomass and making biochar, generally via pyrolysis. The idea here is that you heat biomass to a high temperature in a low-oxygen environment; producing biochar: a chalky black material that's nearly pure carbon.
Biochar is used as a fertilizer and soil additive: it seems like it might improve fertility, increase soil organic carbon (SOC), and reduce soil heavy metal concentrations. For a more detailed overview, see the "Biochar Additions" section of Soil C Sequestration as a Biological Negative Emission Strategy.
Properly produced biochar has the potential to remain durable (keep the C sequestered) in soil for an extended period; however its durability is subject to similar environmental conditions as Soil Organic Carbon, particularly precipitation and soil water content as it relates to microbial decay. It may also have positive effects by reducing soil N2O emissions (another potent greenhouse gas).
Enhanced weathering and carbon mineralization
For a more technical overview of this topic, I highly recommend An Overview of the Status and Challenges of CO2 Storage in Minerals and Geological Formations.
Enhanced weathering – "ex situ"
Common rocks form carbonate minerals when exposed to CO2; often even at atmospheric concentrations; permanently binding the C as a mineral (eg CaCO3 calcium carbonate).
On geologic timescales, this process could restore earth to pre-industrial CO2 levels. The idea behind "enhanced weathering" is to dramatically accelerate this natural process. Project Vesta's website provides an accessible walkthrough to this idea. This is sometimes referred to as "ex situ" mineralization because you're mining rocks and putting them through a sped-up carbon mineralization process aboveground.
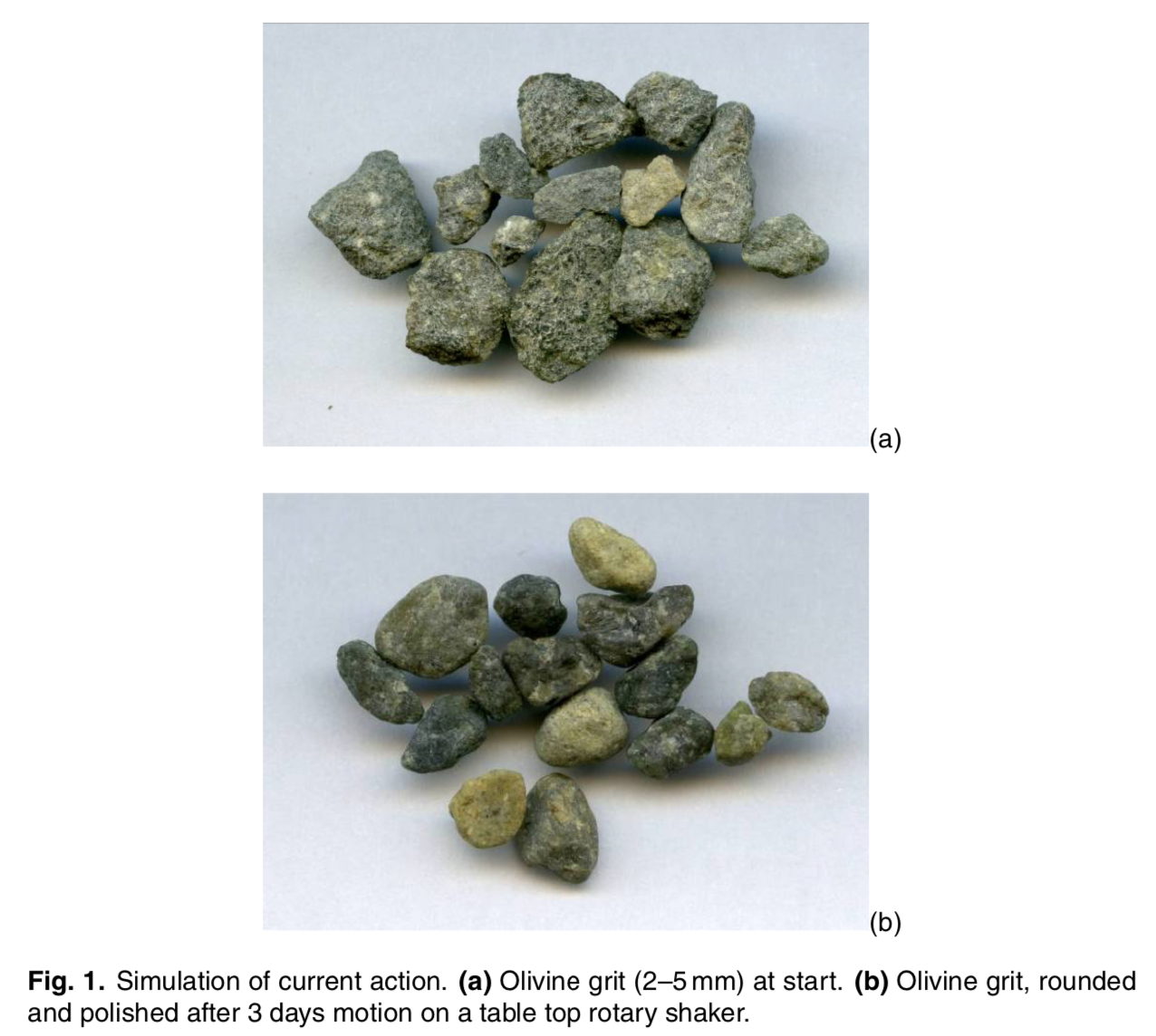
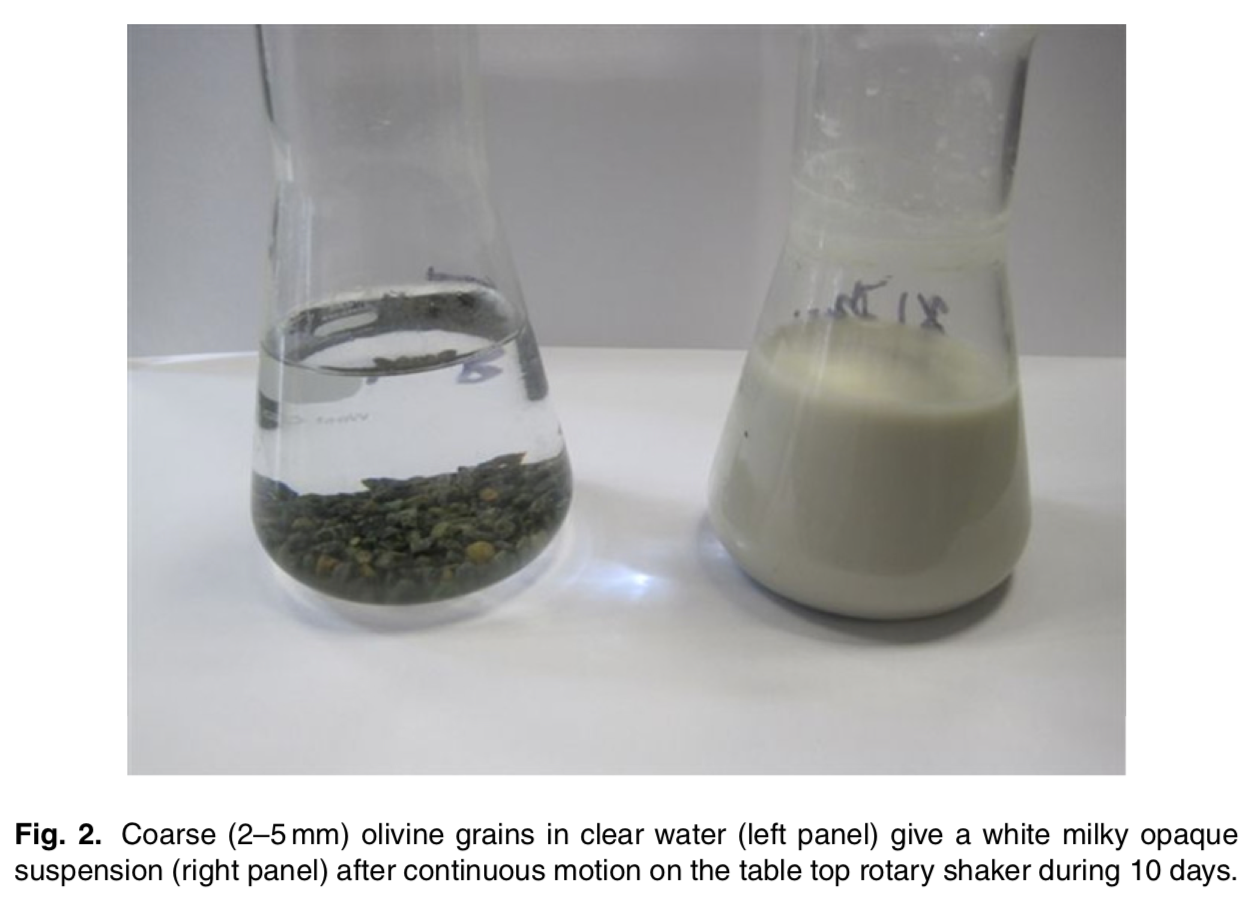
Rocks such as olivine and serpentinite are particularly effective at CO2 binding. The amount of carbon captured is generally proportional to the amount of surface area exposed to air, so we want a low-energy way to grind them to the right particle size to optimally bind carbon. More research is needed into the exact kinetics of the mineralization process, other risks (heavy metal release; ecosystem impacts of distributing vast quantities of small rock particles on land or in oceans; etc); but it's a really interesting idea.
Geologic sequestration - "in situ"
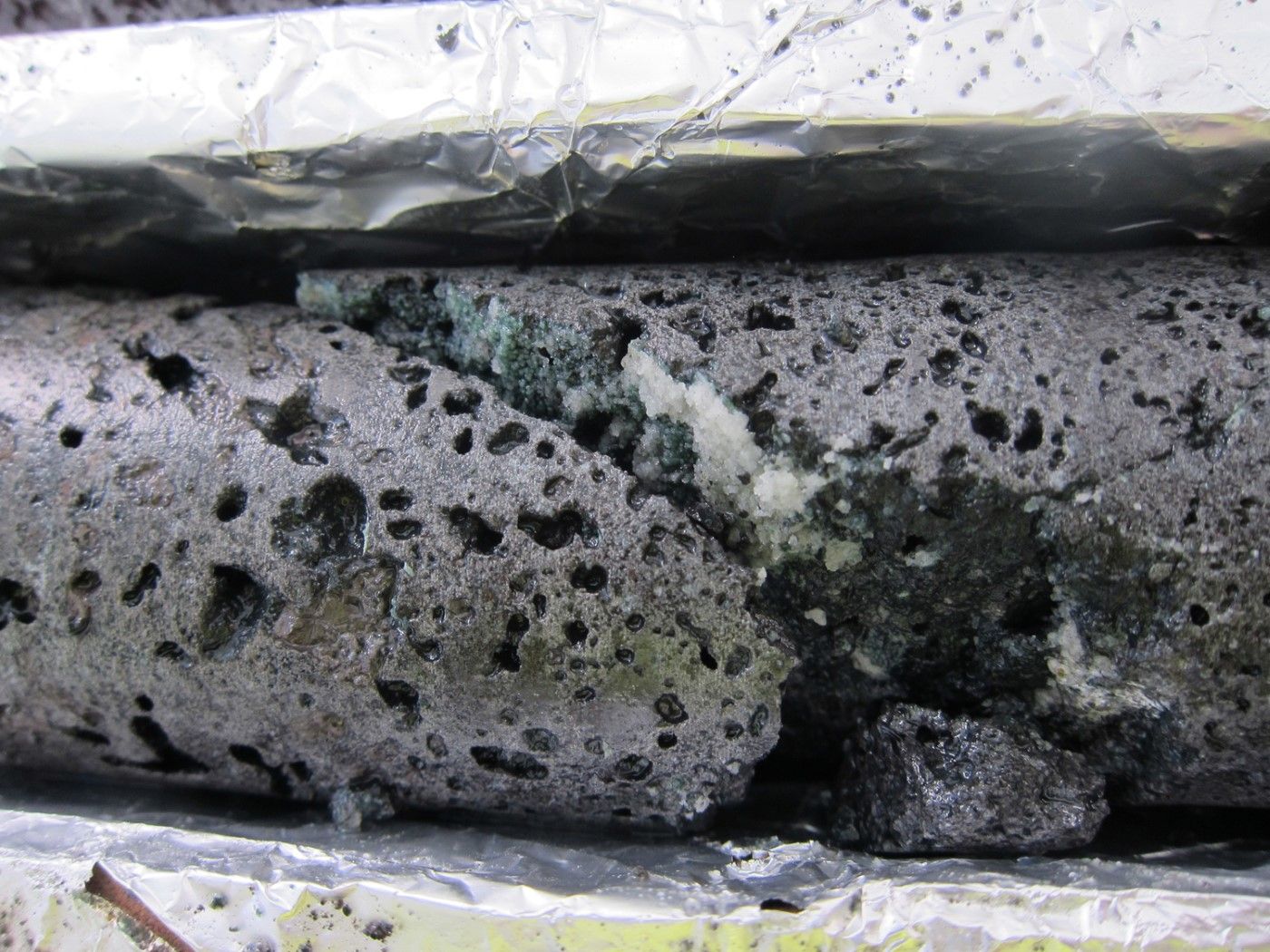
You can think of "in-situ" mineralization as the geologic sequestration component that's crucial to DAC systems.
It happens when you inject a stream of CO2 into the right sorts of geologic formations, and permanently stores the CO2 in a mineral. It's called "in-situ" because you're injecting CO2 to where the rock already is, instead of bringing the rock above ground and exposing it to atmospheric air.
Lifecycle analysis and tradeoffs of carbon removal approaches
It’s important to interpret all carbon removal solutions through a lens of a full lifecycle analysis, which can help answer the question: is the entire operation of a given negative emissions solution net-negative?
For BECCS, for example, this analysis would include the greenhouse gas emissions to grow the biomass, the emissions of building the BECCS plant, the emissions of transporting the biomass to the plant, any loss of CO2 to inefficiencies in the carbon capture system, and the emissions associated with the energy to run the big machine to pump the CO2 underground.
All of this would need to add up to less than the volume of CO2 sequestered -- ideally much less.
Alongside lifecycle analysis, there are a number of dimensions to evaluate carbon removal solutions. Many of these are an oversimplification; but may be helpful to think about the problem space (it’s extremely difficult or sometimes impossible to be sure about many of these questions):
- Efficiency of capture
Does the energy to run a carbon capture machine emit more CO2 than the amount we’re capturing (carbon negative lifecycle)?
How might this change over the coming decades, and how does that affect the cost?
- Permanence of storage
Once it’s captured, what do we need to do to keep it from re-entering the atmosphere? For how long? How much does it cost to do so?
- Ease of measurability/verifiability
Are we sure about exactly how much we’re sequestering?
Are we sure it won’t leak, or we’ll know right away if it does?
Who and how will we penalize if this happens?
- Opportunity cost / moral hazard
What would we use this land for if we didn’t plant a forest on it?
How do we know the next land developer won’t burn it down?
Might this technology slow emissions reduction?
- Potential for scale
What’s the upper bound of how much a CO2 removal technology could sequester? What are the error bars on that estimate?
How much does a given technology cost today? Is there a path for it to be way cheaper in the coming decades?
How might innovation and adoption curves inflect over time; either for this technology directly or in its supply chain?
- Other constraints or tail risks (often risks to permanence or issues exposed in lifecycle analysis)
Does this area have the right geology to support pumping CO2 into the ground?
What happens to this forest if there’s a regime change or land use regulation change?
What if the currency in country X crashes, and farmers need more land?
What if drought/floods caused by a changing climate make this land no longer suitable for the thing we were hoping to do?
What if X technology doesn’t get cheaper as expected/at the expected rate?
Interesting and relevant things I didn't discuss in this post
Here’s a small sample of topics relevant/adjacent to concepts discussed here that I’d like to learn more about. If any of these are particularly interesting to you, tweet (@orbuch) or email (ryan dot orbuch at gmail) me! You can also think of these as requests for blog posts from people with much more expertise in this field:
- Carbon capture and utilization -- point-source capture/traditional CCS, “carbon-to-value”, “air-to-fuels” and more
- Solar radiation management -- stratospheric sulfate aerosol injection and/or marine cloud brightening as a strategy to buy us more time for emissions reduction
- Traditional carbon offsets; their strengths and weaknesses; what we should learn from them to scale negative emissions purchasing
- Ocean fertilization, ocean liming, direct ocean carbon capture, other ocean stuff
- Unit economics and cost/adoption curves of these carbon sequestration options in detail
- The policy and subsidy landscape around carbon sequestration: 45Q; the California LCFS; the European Emissions Trading System, etc.
- Project finance and how it works for negative emissions and energy projects, how it fits with and differs from other funding sources (VC, private equity, tax equity etc)
- Bioengineering approaches: modifying plants to make them better at capturing/storing carbon; engineering proteins for use in DAC sorbents, etc
Conclusion
Here’s what I hope you remember from this piece:
- 10-gigaton-scale negative emissions are necessary in essentially every emissions reduction scenario. We have no choice but to fund, research, and deploy them if we’re serious about keeping warming to 2 degrees; or close to it. We are not even close to on track.
- Negative emissions have been dramatically underfunded in proportion to their importance. This needs to be fixed if we’re going to have a shot at reducing the cost enough to make 10-gigaton-scale deployment possible by midcentury. It will take likely take years or decades for basic research and pilot projects to scale and get cheap enough; so we need to start right now.
- It’s very unlikely any one category of technology, or any one natural approach, will scale enough. We should think of a portfolio across all the approaches outlined here, as well as more I didn’t discuss or have yet to be discovered.
- We face the defining problem of our generation; of the entire human project thus far. Climate spans physics, chemistry, ecology, geology, policy, technology, land use, human rights, and more. It’s time we take this seriously as a gigantic opportunity for human progress, and rally to solve it!
Many people provided feedback on this post, and many more spent time with me over the last few months to help me learn about this field, check my assumptions, and frame my thinking. Thanks in particular to Jeremy Freeman, Christian Anderson, Clay Dumas, Sarah Sclarsic, Peter Reinhardt, Adam Marblestone, Maddie Hall, Klaus Lackner, Nat Keohane, Jennifer Wilcox, Jane Zelikova, Jason Jacobs, April Underwood, Celine Halioua, Florent Crivello, Steve Pacala, Brian Heligman, Michael Nielsen, Jose Luis Ricon, Steve Hamburg, Erika Reinhardt, Alexey Guzey, Ramez Naam, Raylene Yung, Noah Deich, Andrew Bergman, Phil Renforth, Greg Dipple, Giana Amandour, Mason Hartman, Landon Brand, Julio Freedman, Zara L'Heureux, Jeremy Büttner, Nan Ransohoff, Tamara Winter, and many many more than I could mention here!
One of the reasons I’ve had so much fun learning about climate is because the people working on it are really great!